Mars Surface Base—Concept of Operations Proposal
April 14, 2019
The final installment in a three-part series of the unarchival of my old VASTS papers.
Scope Summary
- Need: To possess an extra-terrestrial outpost to support future exploration.
- Goal: Develop a Martian base capable of assessing Martian regolith for fuel and material content that is also capable of extracting fuel and construction materials for testing
- Objective: Effectively convert Mars into a location where a base can self-construct, expand, and support future missions between Earth, Mars, and beyond.
- Mission: Extract carbonates, sulfates, silicones, clays, and deuterium from the Martian soil for base construction materials and rocket fuel.
- Operational Concepts: Three Martian Rovers and a Martian Satellite will be launched to Mars along with the basic components and arrive at the Prometheus base by 2020. These resources will be followed by a skeleton crew in 2030 to establish the rest of the base. These crews will be rotated out of habitancy by crew and supply rockets every two years until a crew of primarily scientists are delivered to the base in 2040. Equipped with the tools for chemical analysis, these scientists will chemically assess the Martian soil until engineers arrive in 2045 for testing. These engineers will design and test applications of Martian soil materials until return launch in 2048 for arrival by 2049.
- Assumptions: All technologies are currently accessible and joint groups can work together efficiently.
- Constraints: Mission launch must occur before 2040 and inhabitants and crew must return to Earth by 2050. Outpost will be a Phase III base consisting of a 10-100-person crew. External factors are public support and funding.
- Authority and Responsibility: Primary authority will fall to the National Aeronautics and Space Administration concurrent with the European Space Agency, SpaceX, and other space agencies. Governmental organization associated with this project will include the NSF and other interested parties.
Mission/Surface Base Name
Mankind has always had, and will possess, the drive to explore and push past and beyond boundaries. This is signified through our constant exploration of new horizons. The most famous explorer, one that even most school children have heard of, is Christopher Columbus. He discovered a completely new continent by pioneering a new route to Asia. The next such infamous explorer is Amelia Earhart, the first female aviator to fly across the Atlantic Ocean. Next came Roald Amundsen the first man to reach the South Pole. These feats were challenged by Yuri Gagarin “the first man to have flown in space” (Tito, 2006). Followed by Neil Armstrong “the first person to step on the surface of the Moon” (National Aeronautics and Space Administration [NASA], 2011). Although future missions followed, this marked the last step of an era of exploration. Since Armstrong’s moon landing, mankind has not landed on a more distant body.
The purpose of this Martian base is to change that fact, the base will be able to house a crew on the Martian surface, making the base the farthest distance a human has been from the Earth. To fit this accomplishment, the name of the base must be similarly impressive. Therefore, this base will be known as the Prometheus Mars Surface Base. Prometheus is a “trickster figure” (Hansen, 2005) accredited with stealing fire from the gods and giving it to humans. In Greek and Roman mythology, this donation is accredited to starting a new age of man, allowing mankind to create cities and vastly improve its condition. Similarly, this base marks a new age of mankind. One where it will be possible to explore Mars and beyond, utilizing resources from the Martian surface to fuel our exploration similar to how Prometheus’ flame helped fuel humanity’s development.
Mission Requirements and System Requirements
The establishment of the Prometheus Martian Base, with the goals of creating an outpost for the extraction of Martian resources for use in future space exploration requires a base suitable for extensive human inhabitation as well as the sophistication to serve its primary purpose. To facilitate human inhabitation, the major components of the base will be located within underground lava tunnels in the Martian surface. Taking advantage of the innate protection offered by the lava tunnels against “radiation, extreme temperature variations, and regolith dust” (Daga et al., 2013), enabling us to forego some shielding. But shielding is still required to protect against residual radiation and provide structural integrity. The interior of the Ice Home, the modules that will make up the common/rest areas and the exercise rooms will consist of a two-story building surrounded by two dynamic layers. The innermost dynamic layer will be filled with a gas that serves to inflate and form a shape for the habitat. The structure will freeze the water within the outer layer in the “-20°C” (Daga et al., 2013) Martian atmosphere providing an outermost layer of frozen water that serves for easy transportation as well as provides structural integrity and radiation protection. The pressurized area of the habitats will be in the shape of an annulus with the center of the annuli being the habitable zones. In the common/rest area Ice Homes, the upper story will consist of individual bed rooms for each inhabitant as well as restrooms and showers. The lower story of the common/rest area Ice Homes will consist of a storage space for food, a dining room, and computers for work and communication. The recreation Ice Homes will be decked with couches, exercise machines, and other activities for the astronauts’ use and enjoyment. The Ice Homes will be deployed to the Martian landing sites and then transported into Arsia Mons where it will be established via the autonomous rovers. For the Ice Home to be habitable, it requires a “predeployed power system” (NASA, 2017) consisting of the numerous solar arrays. The middle, gas containing layer will be filled with the Martian atmosphere, pumped to sufficient “integrated pressure, temperature, and strain” (NASA, 2017). The water to fill the final layer of the Ice Home will originate from the water tanks delivered from the cargo tank. Once the Ice Home’s layers are filled, the integrity of its seams, tubing, and general condition will be tested remotely. After the testing sufficiently proves that the Ice Home is sound, the the astronauts will be launched, and the Ice Home can be furnished. Note that if irreperable damage is detected to the Ice Home, the mission will be delayed until a new Ice Home can be delivered and setup.
The four primary systems that will be utilized in the Ice Home are the Water management interface which consists of a “well-insulated flexible hose with helical heating elements” (NASA, 2017) connected to the heated water supply to prevent the premature freezing of water. The power for the base will be routed through the Ice Homes as they are the primary power receivers with the research lab and rovers at a lower priority state. The power interface of the Ice Home will provide statistics on power production based off of data about the Martian atmosphere. The communications interface within the Ice Home will attach to the external communications array from which communications will be relayed by the satellite in Mars orbit. The Ice Home is 12 meters in diameter, this combined with its two-level design provides “about 198 m2 of floor space (~2131 ft2)” (NASA, 2017) making it suitable for the eventual twenty-five-person crew.
The base will receive the majority of its water content from the Martian ice caps. The extraction will occur prior to the arrival of the skeleton crews and be in a fashion similar to how water is extracted from Earth’s poles. At the South Pole a method known as the “Rodriguez Well” (Przybylski, 2003) is used due to its ability to function in low temperature environments. The Rodriguez Well system can be modified for use in a Martian environment by employing an airtight seal when drilling for water. A Rodriguez Well is created by “drilling [ice] with a hot water sprayer” (Przybylski, 2003). In the case of the Martian poles, the poles consist of frozen carbon dioxide which would still need to be drilled through. Once the hole was a sufficient depth, water would be extracted by “pumping in hot water, and pump[ing] out cool water” (Przybylski, 2003). Mars’ perennial cap consists of two layers, with the top layer consisting of “frozen carbon dioxide about 8 meters thick” (Darling, n.d.) and a bottom layer “made of water ice” (Darling, n.d.). According to Marsis radar data, it is known that there is sufficient water trapped within the ice caps “to cover [Mars] in a liquid layer 11 meter deep” (Darling, n.d.). To tap into this mass of water, the cavity from which water is extracted from must be airtight and maintain a pressure that “minimizes water sublimation” (Hoffman, Andrews, & Watts, 2016). The rover assigned to drilling for water must possess a heated water tank to store and transport the extracted. The water tank for the base’s supply must be approximately 8 m3 large to accommodate the needs of a twenty-five-person crew (assuming that each member consumes 75 gallons of water a day). The primary water storage module would be of aluminum construction to insulate the water within and maintain its liquid state.
In terms of energy, the Martian base will be powered primarily by solar power. Arsia Mons, the proposed location of the Prometheus Martian base, is a equatorial shield volcano. Due to its proximity to the equator, it will be able to sufficiently absorb energy from the sun to power the base day and night. By combining the numerous solar arrays with a high-efficiency battery system capable of numerous charge cycles, the base can maintain near constant power. Although power needs will not be large during the inhabitance of the skeleton crews, the larger crew will certainly require additional power. To mitigate the problems that solar cells face in the Martian atmosphere, including “suspended atmospheric dust” (Landis, Kerslake, Jenkins, & Scheiman, 2004), “low operating temperatures” (Landis et al., 2004), and the deposition of dust on the solar array, the solar cells used will be optimized for Martian wavelengths, be arranged in a tent structure, and be heated. These modifications will make cleaning and maintaining the solar cells simpler as well as promote energy efficiency.
To provide a mode of transport on the Martian surface, three rovers will be utilized. The light vehicle for the majority of crew operations will be a modified Apollo Lunar Roving Vehicle (LRV) optimized for the Martian surface. Although the original LRV had a mass of “210 kg” (Zakrajsek et al., 2005) and could manage a payload of “490 kg on the lunar surface” (Zakrajsek et al., 2005) due to its “DC series wound 190 w motors” (Zakrajsek et al., 2005), the modified LRV would utilize a pair of “three phase, four pole AC induction” (Tesla Motors, n.d.) motors capable of providing 416 horsepower. Arranged through a system of three locking differentials, each wheel would receive its own power providing traction and stability on the Martian regolith. A larger rover would be an Antarctician Nomad Rover for cargo purposes. This rover is highly suited for the Martian surface due to its “four aluminum wheels with cleats provid[ing] traction in soft sand” (Zakrajsek et al., 2005). The third, and final, rover will be utilized to transport large volumes of astronauts and be utilized extensively in geologic studies. Designed after Rodger Arno’s planetary surface vehicles (Arno, 1999) this rover is designed for heavy-duty autonomous use. Built into its design are the capabilities of carrying “500kg of plowing, digging, and drilling equipment” (Zakrajsek et al., 2005) as well as “manipulator arms that can lift up to 50 kg” (Zakrajsek et al., 2005). All rovers will possess autonomous capabilities in the initial setup of the Martian base. Additionally, they will all have positioning systems and communications arrays for communication to the Ice Home and to mission control.
Communication with the Prometheus Martian base, especially communications suitable for human missions, will require a high bandwidth connection that enables astronauts to telecommunicate with their families and with mission control. The primary infrastructure the Prometheus Martian base’s communications will build upon are NASA’s Deep Space Network (DSN); a “network of antennas” (NASA, n.d.b) utilized for “interplanetary spacecraft missions” (NASA, n.d.b). Although NASA’s DSN can communicate with Martian rovers, a superior system must be developed for human inhabitation. For this to occur, the Prometheus base’s communications array will have three parts: a base communications array, a trio of in-orbit satellites, and a L5 Lagrange point satellite. The surface communications array will be WLAN array for surface communications and a S-band array for satellite communications both mounted on a “deployable 10-meter boom” (NASA, 2007) with a built-in thermal control system of “radiators, heat pipes, cold plates, MLI, heater, [and] thermocouples” (NASA, 2007). This surface communications array will communicate with the trio of satellites in Martian orbit, which will then communicate with the satellite in the L5 Lagrange point, which will finally communicate with Earth’s DNS system. This setup will allow for communications around the Sun enabling communications throughout the mission.
Due to the need to chemically assess the Martian regolith, devices and tests must be included within the mission. The device used to gather Martian regolith samples is known as the Regolith Advanced Surface Systems Operations Robot (RASSOR) which utilizes “digging bucket arms at the ends of [its] body that rotate in opposite in directions” (NASA, n.d.c). The dual bucket arms enable the RASSOR to “step and climb over obstacles” (NASA, n.d.c) enabling the autonomous deployment of these devices to categorize the Martian regolith. The tests that will be applied to Martian soil to categorize its properties include thermal analysis consisting of a “resistive heater of nichrome wire windings” (Smrekar et al., 1999), laser spectroscopy via a “tunable diode laser” (Smrekar et al., 1999), and the assessment of physical parameters of “particle size, packing density, [and] salt cementation” (Smrekar et al., 1999) through heat distribution.
The air and water filtration systems will utilize a system of higher plants and microalgae spirulina to satisfy the oxygen, water, and some of the food needs of the crew. The algae serve to satisfy the oxygen and water needs of the crew by absorbing carbon dioxide and water to produce “0.60 kg algae (dry weight)” (Tikhomirov et al., 2007) every day. The higher plants will similarly recycle air as well as serve as a greater food source for the crew. This system can also recycle human waste by submitting waste to a physico-chemical incineration producing carbon dioxide, water, and “subsidized organics” (Tikhomirov et al., 2007) all of which are given to the algae as nutrients. The algae are then able to resupply this waste as biomass and minerals which the higher plants harness from the soil and nutrient solution that they are subject to.
Mission Timeline
This timeline will be the approximate guidelines the mission will follow to ensure the proper conduction of base operations. It is recognized that Mars approaches nearest to Earth every two years and approximate travel time is 300 days. The same cargo rockets, both five-man crew/cargo rockets, and large capacity crew rockets are recycled throughout the mission with refueling on Earth.
Date | Event |
---|---|
October, 2020 | Three satellites are launched for Martian orbit to further categorize lava tunnels and serve as a communications relay. |
October, 2021 | Satellites are in Martian orbit and are orbiting Mars. Satellites work together to maintain constant contact with the L5 Lagrange point satellite. |
October, 2022 | First cargo rocket sent to Martian landing site carrying a modified Antarctician Nomad Rover, the Arno Rover, the Ice Home, and the water and air tanks. |
October, 2023 | Rocket has landed on landing site and the materials are in-transit to the lava tunnels. Materials will then be prepared for human inhabitation. |
October, 2024 | Second cargo rocket is delivered to Mars containing one of four solar arrays, the advanced communications module, the water/air recycling plant, and the polar water extractor. |
October, 2025 | Solar array, communications module, and recycling plants are setup and incorporated into Ice Home. Base can currently support life. |
October, 2026 | The third cargo rocket is launched carrying two more solar arrays, the inflatable lab, Martian suits, and a modified Apollo Lunar Roving Vehicle (LRV) suitable for Martian use. |
October, 2027 | The third cargo rocket lands on the Martian surface and materials are transported from the landing site to the Prometheus base. |
October, 2028 | A crew rocket carrying five crew members, the final solar array, and delicate instruments such as microscopes, scales, freezers, and cabinets are also carried Tests are carried out on current base components to ensure integrity and safety. |
October, 2029 | Crew rocket arrives on Martian surface and is transported to Prometheus base. Setup begins to develop lab and further base design. |
October, 2030 | Second crew rocket with five members is launched equipped with common area and exercise equipment. |
October, 2031 | Base undergoes setup and now consists of ten members. These astronauts will setup the common areas and exercise equipment. |
October, 2032 | Third crew rocket is launched carrying high capacity food stuffs and chemicals and beakers required for scientific study. Crew rocket on Mars is launched for return carrying original five-man crew. |
October, 2033 | Original five-man crew returns to Earth. Third crew rocket lands on Martian surface and is transported to Prometheus base. Chemicals and equipment are inventoried and assessed for damage. |
October, 2034 | Fourth crew rocket is launched carrying materials for survey and high-density mapping. Second crew is launched back to Earth. |
October, 2035 | Fourth crew rocket lands and surveying of Martian soil begins, basic chemical experiments are conducted. Second crew returns to Earth. |
October, 2036 | Fifth crew rocket is launched carrying a secondary Ice Home, a pair of solar arrays, and another water/air recycling system. Third crew is launched back to Earth. |
October, 2037 | Fifth crew rocket lands and Ice Home, solar arrays, and recycling system are setup. Systems are tested for high-occupancy |
Fourth crew is launched back to Earth. | |
October, 2038 | Large crew rocket is launched carrying team of seven astronauts and eight scientists along with personal effects and additional food stuffs. Fifth crew is launched back to Earth. |
October, 2039 | Large crew rocket lands on Martian surface carrying fifteen-man crew and scientists and astronauts work concurrently to obtain mars soil samples Fifth five-man crew returns to Earth . |
October, 2044 | A crew of ten engineers will be launched to Mars to begin testing. This rocket will contain major engineering equipment including 3D printers and other design tools. |
October, 2045 | Engineers, scientists, and astronauts work to gather soil samples from assessed locations to extract resources and develop a resource map of the Martian surface. Engineers work to develop machines and technologies for the extraction of resources. |
October, 2048 | All crew board rockets they were delivered on. |
October, 2049 | All crew returns to Earth. |
Outpost Location
The problems that astronauts will face in a Martian environment include dust storms, solar wind, a toxic atmosphere, cosmic radiation, and extreme temperatures, all of which make un-shielded humans less than optimal for this mission. A suitable habitat for this mission would need to shield humans from all of these factors as well as be suitably designed to minimize weight to decrease launch costs. Therefore, a potential solution to the habitat issue would be to utilize lava tubes on the Martian surface. Lava tubes are naturally formed caverns created by lava flows that have “over-crusted to form subsurface flowing rivers of lava” (Daga et al., 2013). The tubes offer “protect[ion] from ultraviolet radiation…[and] constant temperatures…[that] permit liquid water to persist” (Daga et al., 2013). As the lava that formed the over-crust drains away, it leaves a cave. By analyzing the “sinuous rillies represent[ing] lava tubes” (Daga et al., 2013), it was found that these lava tubes were significantly larger than “terrestrial lava tubes” (Daga et al., 2013). The presence of such protective elements decreases the required complexity of the base, enabling higher efficiency in the launch system. Additionally, by inhabiting the lava tube, the Martian base forgoes the “need for water (as a potential radiation shield)” (Daga et al., 2013), enables the use of “electronics and test instruments that are sensitive to radiation” (Daga et al., 2013), can maintain “a benign -20°C” (Daga et al., 2013), and “limit the intrusion of dust” (Daga et al., 2013). Therefore, by locating the entire Martian base within such lava tubes, the base design can become simplified, the costs can be lowered, and the crew can enjoy greater safety.
Such lava tubes have been located by the Mars Odyssey Thermal Emission Imaging System (THEMIS) which located “potential skylight openings” (Cushing, Titus, Wynne, & Christensen, 2007) on Arsia Mons, a shield volcano in Mars’ southern hemisphere. THEMIS was able to differentiate the lava tunnels from impact craters due to the detection of “collapse pits” (Cushing et al., 2007) rather than “raised rims or eject patterns” (Cushing et al., 2007) traditionally indicating impact craters. The lava tunnels are able to maintain a smaller variance in temperature than normal displaying greater “nighttime temperatures…than all nearby surfaces” (Cushing et al., 2007) indicating the maintenance of heat. It was additionally found that the lava tunnels were rich in “carbonates, sulfates, silica, and clays” (Leveille & Datta, 2010) essential to the construction of further structures, rocket fuels, and solar panels. If the Prometheus Mars Surface Base is located within these tunnels, the base will be able to maintain a high degree of astronaut safety and due to the location’s proximity to the equator, the surface of Arsia Mons will be exposed to sunlight for a large amount of time, enabling the use of solar panels as a primary power source of the base.
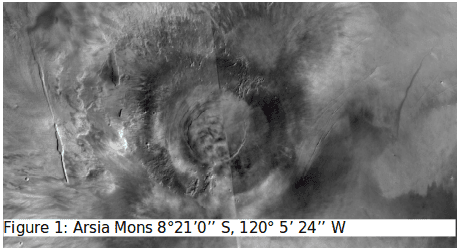
As seen in Figure 1, Arsia Mons is located in proximity to the equator and is spotted by seven proposed skylights as indicated in Figure 2.
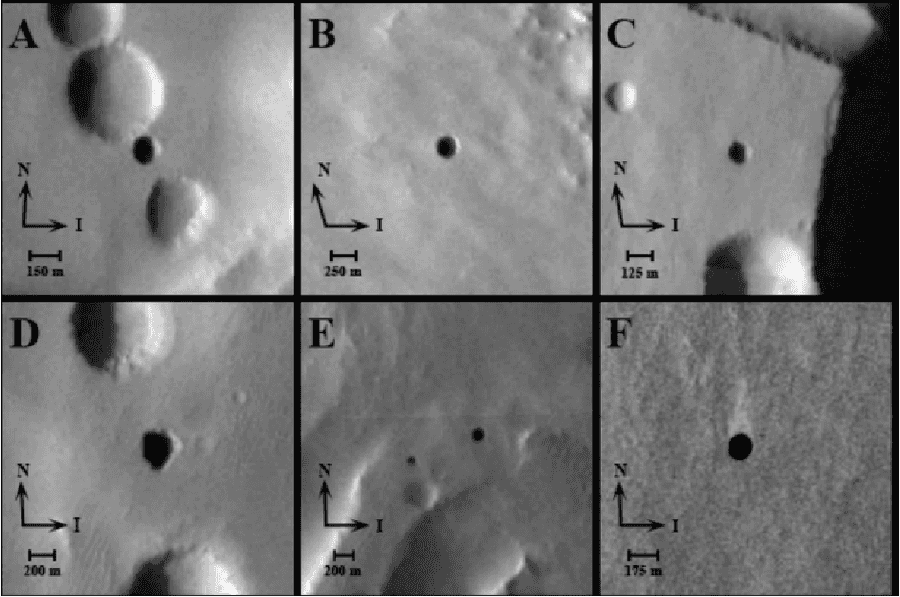
Clockwise from upper left, the pits are named “Dena, Chloe, Wendy, Annie, Abbey, Nikki, and Jeanne” (Cushing et al., 2007). The skylights are located at [6.084°S 239.061°E], [4.296°S 239.193°E], [8.099°S 240.242°E], [6.267°S 240.005°E], [8.498°S 240.349°E], and [5.636°S 241.259°E] respectively.
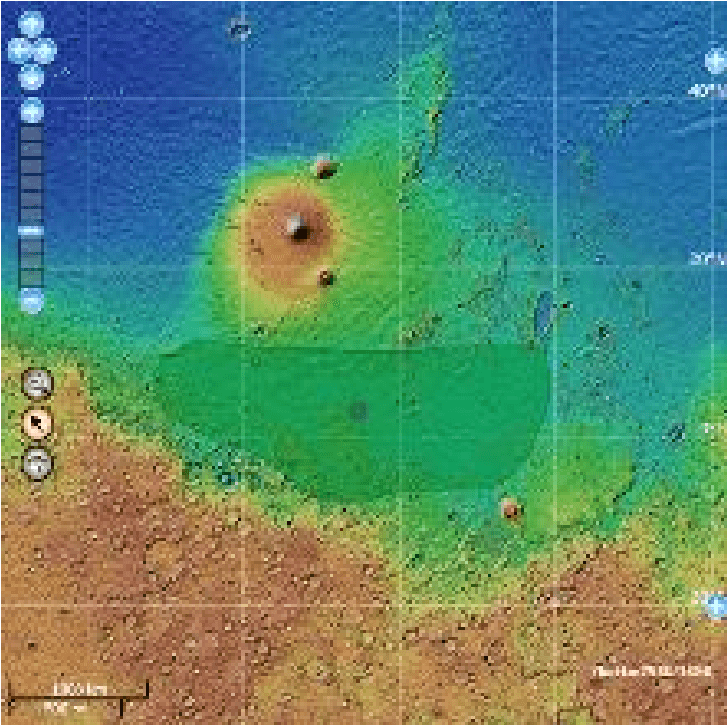
Martian landings will occur on either the 3000-kilometer diameter Elysium Planitia (Figure 3) at “2.98°N and 154.74°E” (Gazetteer of Planetary Nomenclature, 2006) or the 1000-kilometer diameter Meridiani Planum (Figure 4) at “0.04° S and 356.86° E” (Gazetteer of Planetary Nomenclature, 2018). Both are known for their low elevation, flatness, and proximity to the equator making them suitable for landings.
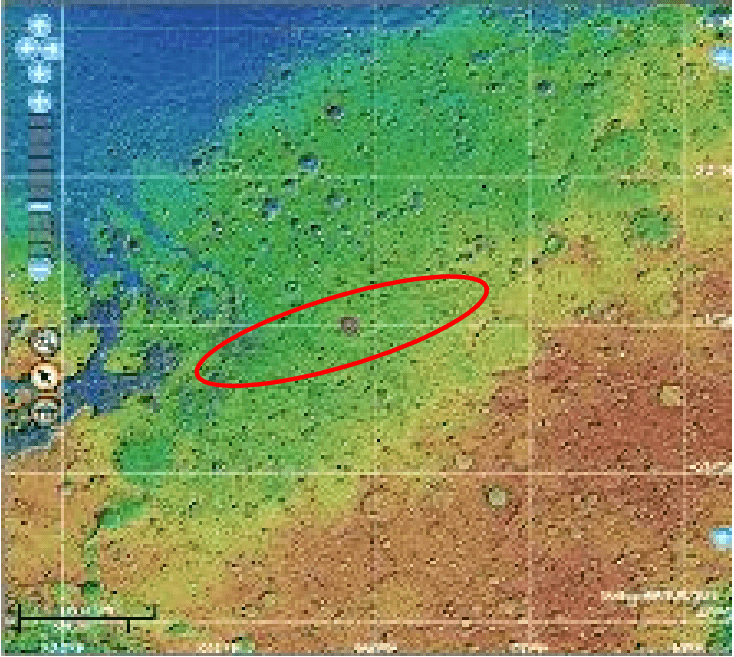
Mission Constraints
Mission constraints that can be addressed internally, through proper planning and decision-making, are the limitations on mission launch (to be prior to 2040), the creation of a Phase III base, and departure from Mars for Earth return by 2050. These constraints are addressed by having the base with an eventual twenty-five-member occupancy after years of wavering between five and ten crew members. To accommodate for the 2040 start date and 2050 end date, astronauts are launched well in advance.
The other largest limiting factor for this mission will be funding received from the government. This single factor limits the duration, degree of technological advancements, and scale of this project. A decrease in funding could lead to shorter training times among astronauts, a lower number of supplies, lower frequency of launches, a smaller base, and a shorter mission duration. By having sufficient funding, the mission could easily fulfill its goals of maintaining the next human outpost and representing the next frontier of human exploration, establishing a symbol of humanities prowess for ages to come. To guarantee this high degree of funding, the public, and therefore policy-makers must be on board with the mission. This includes extensive advertising prior to the mission start, high-investments in public-benefitting technologies, and having a transparent portal through which the public can view the effects of their funding. But the funding constraint can only be overcome by emphasizing the direct affects of the mission as well as its significance.
Another constraint that limits resource delivery and the complexity of the mission are the delivery windows to the Martian surface. Mars reaches its closest distance to Earth once every two years requiring the even number launches as seen in the timeline above. Additionally, the commute time to Mars is approximately 300 days resulting in a high lag time between the communication between astronaut’s need and delivery. Therefore, astronauts will be required to plan significantly ahead and maintain constant stock of resources contained within the base. In doing so, a scarcity can be avoided, and astronauts can maintain their safety.
The final constraint that affects the mission is the transmission abilities of the communications system. In high interference situations, such as on the dusty Martian surface, the capabilities of communications systems will be significantly decreased. The interference that exists in surface to surface communications as well as surface to satellite communications can cause portions of surveying to slow down as astronauts run the risk of losing communication and becoming lost. Another communications limitation is when the Mars becomes the greatest distance from the Earth. This can lead to massive lag times and low bandwidth communications causing a overall decrease in quality of communications.
Risks/Dangers
The primary hazard astronauts will experience throughout their mission is radiation. Radiation encompasses all energy that is transmitted in the “form of rays, electromagnetic waves, and/or particles (NASA, 2008) encompassing radio waves with a wavelengths of “1 meter and greater” (NASA, 2008) to gamma rays with wavelengths of “10-12 [meters] and smaller” (NASA, 2008). The radiation that is damaging to humans is high-energy, low wavelength waves mostly “x-rays, gamma rays, and…solar particle events” (NASA, 2008). Although the Earth offers protection from those types of radiation with the magnetic field and “Earth’s atmosphere” (NASA, 2008), as we leave the protective habitat of the Earth, this protection gradually fades until it is non-existent such as in lunar orbit or in-transit to Mars. The two primary modes of radiation that will affect this mission are ionizing and non-ionizing radiation. Non-ionizing radiation is the traditional form of weaker photon-based radiation such as “radio frequencies, microwaves, infrared, visible light, and ultraviolet (UV) light” (NASA, 2018.a). Ionizing radiation is all non-photon radiation and high energy photon radiation including “alpha particles, beta particles, gamma rays, x-rays, and galactic cosmic radiation (GCR)” (NASA, 2018.a). Ionizing radiation is the more dangerous form of radiation due to its ability for “mov[ing] through substances and alter[ing] them” (NASA, 2018.a) ionizing atoms “in the surrounding material” (NASA, 2018.a) as the particle passes through. Due to the nature of ionizing radiation, it is more damaging to astronauts than other forms of radiation. Alpha rays, a part of ionizing radiation, “cause 20 times the damage” (NASA, 2008) of x-rays and gamma rays and are therefore, given a higher weight when calculating tolerable radiation doses. Additionally, it is assumed on a 3-year Mars mission, “about 30% of cells in the body” (Setlow, 2003) due to both direct impact from ionizing radiation as well as being affected from “neighboring cells that have been hit” (Setlow, 2003). This radiation can increase an astronaut’s risk to mutations as well as alter the immune systems’ ability to protect the body, increasing the bodies susceptibility to cancers as well as the traditional cold and flu.
Due to radiation being a risk that is innate in space exploration, especially in a Martian mission, a blend of operational and engineering countermeasures must be considered to combat radiation. Operational countermeasures include the duration a astronaut can be “a radiation worker in space” (NASA, n.d.a), assigning “appropriate mission lengths” (NASA, n.d.a), as well as incorporating a “radiation safe haven” (NASA, n.d.a) within the spacecraft. Engineering countermeasures include incorporating “hydrogen rich shielding” (NASA, n.d.a) such as polyethylene in base design as well as creating a “insulating layer of [Martian] soil (regolith) or water” (NASA, n.d.a) as a radiation buffer. In this mission, the spacecraft will feature extensive use of polyethylene throughout its design as a radiation buffer and be able to protect astronauts from the majority of the radiation experienced in the mission. On the Martian surface base, crew will be protected by the lava tunnels the base is located in as well as the rovers and suits that feature radiation protection as well. A constant throughout the mission will be the dietary measures offered to astronauts. Dietary measures will include “antioxidants” (NASA, n.d.a), “pectin fiber” (NASA, n.d.a), and “omega-3-rich fish oils” (NASA, n.d.a). All of these can prevent or reduce radiation damage by “soaking up radiation produced free-radicals” as well as increase the rate of removal of “radioactive substances…from the body” (NASA, n.d.a).
Microgravity is another high-priority risk that is posed to astronauts in varying degrees throughout the mission. In space, the body experiences what is known as microgravity where the body experiences very small amounts of gravity. On other planets, the body experiences reduced gravity. Mars’ gravity is only “0.377” (NASA, 2018.b) of Earth’s gravity. Both of these states of gravity result in varying degrees of atrophy within the human body. In weightlessness, at least, the body experiences what is known as “space motion sickness” (Virginia Space Grant Consortium [VSGC], n.d) where the body must learn to reinterpret special cues without the normal cues from the vestibular system. Although this condition subsides after “a few hours or days” (VSGC, n.d) in the microgravity environment, there are more permanent effects from the microgravity environment. These effects include the “deconditioning of physiological systems” (VSGC, n.d.a) including the cardiovascular system and the musculoskeletal system. In space and on Mars, the lack of the magnitude of resistive force applied by the Earth’s gravity results in the “loss of calcium, nitrogen, and phosphorous” (VSGC, n.d.a) and “decreased bone size and volume” (VSGC, n.d.a). Additionally, the loss of the Earth’s gravity can lead to “weakened reflexes, and a decreased tolerance for physical work” (VSGC, n.d.a) this has obvious implication when astronauts are required to work in a Martian environment after years of microgravity transit as well as survive in the higher-gravity environment of the Earth upon return.
A method of mitigating the effects of microgravity in-transit is to incorporate exercise machines into the space craft and base as well as exercise time into the astronauts’ daily schedules. This would prevent the damage to the cardiovascular and musculoskeletal systems experienced throughout the mission. Limitations to this solution are the amounts of time available for each astronaut to exercise, primarily dependent on the responsibilities of the astronauts, the number of astronauts, and the number of exercise machines. On the surface, a rehabilitation program could be utilized by all crew members to acclimate themselves to the Martian gravity. Another method of reducing the effects of microgravity, and therefore the rehabilitation period, is to increase the velocity at which astronauts travel to Mars, decreasing the time spent in the microgravity environment. Due to the “45-day ‘reconditioning period,’ to build up bone and muscle strength” (Wall, 2016) employed by astronauts returning, to Earth, a similar process could be utilized on Mars. To alleviate the time-draw of this acclimation period, the mission could have a greater duration, making the acclimation period appear shorter in relativity.
Other risks inherent in the mission design are launch risks, meteoroid impact, and the presence of a toxic environments. Although each of these events are of the criticality one risk category, they are preventable through careful planning and training. Launch risks are inherent to any space mission. The very act of launching a spacecraft itself is very complex requiring the cooperation of multiple groups and people. But, as NASA matured and grew into the organization it is today, it was able to properly decrease launch risks to appropriate levels. Launch risks can be alleviated by enacting a decision-making board that is free from group-think and can therefore, make decisions without a concurrence-seeking tendency. In-transit, asteroid impact can be avoided by planning and timing the route to Mars so that it avoids known asteroid routes. On the Mars surface base, the risk of meteoroid impact is lowered due to the presence of the Martian atmosphere. Additionally, the Martian base will be sheltered by the lava tunnels which are long-standing caves with a low chance of collapse in the face of a meteoroid impact. The in-transit presence of the vacuum of space will be present indefinitely. Astronauts recognize the risk of having nothing protecting them except for the spacecraft. But, the success of numerous space missions as well as the International Space Station (ISS) near guarantees safety from the vacuum of space. To prepare for the toxic Martian environment, astronauts will be trained in the proper safety protocols to ensure the safety of the crew as well as the integrity of the base. These risks represent a very small minority of risks that cannot be wholly addressed, the majority of dangers faced by the astronaut crew have been resolved in previous missions this includes maintaining the proper atmosphere for astronauts, creating high-integrity space suits, and, to some extent, having high-reliability launches.
Crew Responsibilities and Functions
The primary activity for the astronauts inhabiting the Prometheus Martian Base is to develop and maintain the life support systems throughout the base. This includes the production of water, electricity, as well as the recycling of air and water. In terms of water and air recycling, astronauts will be required to maintain and monitor the system until it is stable requiring the addition of daily supplements and massive amounts of time in terms of its upkeep. This maintenance will be the primary responsibility of both the skeleton crews and the full crew as it is the main life-support system that is utilized throughout the base. The other system requiring constant maintenance will be the electrical system. The electrical system will require constant monitoring, cleaning, and repair requiring a electrician present throughout the duration of the mission to maintain the electrical systems.
As Martian geology is of high-interest to the Prometheus Martian base, astronauts will be expected to be able to analyze and assess the Martian soil. By undergoing training in RASSOR use prior to arrival at the Prometheus Martian base, astronauts will be able to effectively categorize the Martian soil and map locations of interest on the Martina surface. Additionally, if the skeleton crew briefly analyzes the soil, the full crew can be more efficient in their extraction of materials and further classification, building on data extracted by the skeleton crew. In addition to Martian soil research, astronauts will be dedicated to side projects including astronomy, biochemistry, and cell biology to develop our understanding of the Martian environment.
Crew recreation will occur through the recreation Ice Home. This Ice Home will contain board games, to telecommunication devices, to exercise machines, enabling astronauts to spend their free time in their chosen method. In terms of entertainment, the Ice Home will be supplied with a TV, a computer, and numerous games for the astronauts to destress with. The astronauts will also be given the capability to communicate with their families on Earth through the Ice Home’s communication module during this leisure time. In terms of exercise, the Ice Home will be equipped with compression treadmills, a bench-press machine, and a leg press machine to enable astronauts to maintain their physical state. Each astronaut will also be assigned a psychological and physiological supervisor to monitor their eating, work, and exercise habits.
The skeleton crews will consist of an electrician, a medic, an engineer, a pilot, and a surveyor. The full crew will be of a similar composition with the seven astronauts modeling a skeleton crew while the remaining eighteen scientists and engineers are secondarily astronauts. The scientists will range from geophysical to environmental scientists concentrated primarily on studying the chemistry of the Martian regolith. The astronauts will employ a plethora of skills with a wider range than that of the skeleton crew including a physicist and a research supervisor. The engineers will range from chemical engineers to hardware engineers. These ranges of careers covered by the crew focus around Martian geology but not all of them are dedicated specifically to it, enabling diversity of ideas and methods throughout the crew.
Crew selection will occur to emphasize diversity in ages, sex, and nationalities. Crew selection will be designed to emphasize the strengths of each crew member as well as create a crew that works flawlessly as a unit. Therefore, crew members will not only be selected for their innate “duty responsibility, self-control, and restraint” (Sgobba, et al., 2018) formulating an astronaut that is dedicated to the mission and can ensure its success. Educationally, the astronaut must have “at least a bachelor’s degree from an accredited institution” (VSGC, n.d.b). Astronauts will be selected to be aged between 26 years old and 46 years old to ensure a diversity of experiences and abilities.
References
Cushing, G. E., Titus, T. N., Wyne, J. J., & Christensen, P. R. (2007). Themis Observes Possible Cave Skylights on Mars. Lunar and Planetary Science. Retrieved from https://www.lpi.usra.edu/meetings/lpsc2007/pdf/1371.pdf
Daga, A., Allen, C., Battler, M. M., Burke, J. D., Crawford, I. A., Leveille, R. J…Tran, L. T. (2013). Lunar and Martian Lava Tube Exploration as Part of an Overall Scientific Survey. Planetary Sciences Decadal Survey. Retrieved from https://www.lpi.usra.edu/decadal/leag/AndrewWDagaFINAL.pdf
Gazetteer of Planetary Nomenclature. (2006). Elysium Planitia. Retrieved from https://planetarynames.wr.usgs.gov/Feature/1784
Gazetteer of Planetary Nomenclature. (2018). Meridiani Planum. Retrieved from https://planetarynames.wr.usgs.gov/Feature/3854?__fsk=-269173990Tito, D. (2006). Yuri Gagarin. Retrieved from https://en.wikipedia.org/wiki/Yuri_Gagarin#cite_note-time2006-3
Hansen, W. (2005). Classical Mythology: A Guide to the Mystical World of the Greeks and Romans. Oxford University Press.
Landis, G. A., Kerslake, T. W., Jenkins, P. P., & Scheiman, D. A. (2004). Mars Solar Power, Second International Energy Conversion Engineering Conference. Retrieved from https://ntrs.nasa.gov/archive/nasa/casi.ntrs.nasa.gov/20040191326.pdf
Leveille, R. J., & Datta, S. (2010). Lava Tubes and Basaltic Caves as Astrobiological Targets on Earth and Mars: A review. Planetary and Space Science. 58, 592-598. Doi: 10.1016/j.pss.2009.06.004
National Aeronautics and Space Administration. (2018.a). Why Space Radiation Matters. Retrieved from https://www.nasa.gov/analogs/nsrl/why-space-radiation-matters
National Aeronautics and Space Administration. (2018.b). Planetary Fact Sheet-Ratio to Earth Values. Retrieved from https://nssdc.gsfc.nasa.gov/planetary/factsheet/planet_table_ratio.html
National Aeronautics and Space Administration. (2017). Ice Home Mars Habitat Concept of Operations. Retrieved from http://bigidea.nianet.org/wp-content/uploads/2018/07/IceDome-ConOps-2017-12-21v-reduced.pdf
National Aeronautics and Space Administration. (2011). Project Apollo: Astronaut Biographies. Retrieved from https://history.nasa.gov/ap11ann/astrobios.htm#Armstrong
National Aeronautics and Space Administration. (2008.a). Space Faring: The Radiation Challenge. Retrieved from https://vsgc.spacegrant.org/course/pluginfile.php/29115/mod_assign/intro/RadiationPrimer.pdf
National Aeronautics and Space Administration. (2007). NASA’s Lunar Communications & Navigation Architecture [PowerPoint Slides]. Retrieved from https://www.nasa.gov/pdf/203072main_LAT2%20C-N%20to%20ESTO%20TEC%202007-11-15%20rev2.pdf
National Aeronautics and Space Administration. (n.d.a). Space Radiation. Retrieved from https://www.nasa.gov/sites/default/files/atoms/files/nasa_space_radiation_ebook_0.pdf
National Aeronautics and Space Administration. (n.d.b) About the Deep Space Network. Retrieved from https://web.archive.org/web/20120608040231/http://deepspace.jpl.nasa.gov/dsn/
National Aeronautics and Space Administration. (n.d.c) Engineers Building Hard-working Mining Robot. Retrieved from https://www.nasa.gov/topics/technology/features/RASSOR.html
Przybylski, G. (2003). Water at the South Pole. Retrieved from http://glacier.lbl.gov/gtp/SouthPole/rod_well.html
Setlow, R. B. (2003). The hazards of space travel. European Molecular Biology Organization. 4(11), 1013-1016. Doi: 10.1038/sj.embor.7400016.
Smrekar, S., Catling, D., Lorenz, R., Magalhaes, J., Moersch, J., Morgan, P.,…Blaney, D. (1999). Deep Space 2: The Mars Microprobe Mission. Journal of Geophysical Research, 104, 27013-27030. Retrieved from http://citeseerx.ist.psu.edu/viewdoc/download?doi=10.1.1.707.9964&rep=rep1&type=pdf
Tesla Motors. (n.d.). Model S Specifications. Retrieved from https://www.tesla.com/support/model-s-specifications
Virginia Space Grant Consortium. (n.d.a). Microgravity: Zero g and Mars g. In Module 6: Human Mission to Mars. Retrieved from https://vsgc.spacegrant.org/course/mod/book/view.php?id=6312&chapterid=10180
Virginia Space Grant Consortium. (n.d.b). Lunar Exploration Science. In Module 3: Designing for Space. Retrieved from https://vsgc.spacegrant.org/course/mod/book/view.php?id=6297&chapterid=10084
Zakrajsek, J. J., McKissok, D. B., Woytach, J. M., Zakrajsek, J. F., Oswald, F. B., McEntire, K. J.,…Goodnight, T. W. (2005). Exploration Rover Concepts and Development Challenges. Retrieved from https://www.nasa.gov/sites/default/files/atoms/files/exploration_rover_concepts_grc.pdf
Appendix
Outreach Page
The Prometheus Mars surface base marks the new frontier of human exploration. Although it has been many years since man has explored a new planet, this latest instalment of the National Aeronautics and Space Agency (NASA) is a monument to human perseverance. In an international collaboration, NASA hopes to assess the Martian soil for future use in further construction of the Martian base and eventually the establishment of a permanent, self-sustaining settlement. The Prometheus Martian surface base marks the first step toward that journey.
The Prometheus Martian surface base features a state-of-the-art solar power system, a revolutionary water extraction technology, an innovative resource recycling system, and three rovers which will be named by the public. With the launch date for the mission set to October of 2020, we hope to recruit and employ numerous astronauts focused in the earth science and chemistry fields. Although the first ten years of the base will be run by a skeleton crew consisting of the bare minimum of personnel to run the base, the base will eventually be staffed by twenty-five astronauts, scientists, and engineers, all experts within their fields. The goal for this base is to analyze the Martian soil for eventual resource extraction leading to the enabling of construction of future base elements and the fueling of our rockets. The Martian soil is known to contain deuterium—a proposed rocket fuel—as well as clays, silicones, and carbonates, all essential to the construction of further elements of the base.
As an international cooperation, it is expected that this base marks a new era of human cooperation and piece. Gone are the days of the Cold War where competition reigned supreme. Instead, we know seek cooperation for the sake of greater innovation and progress for a brighter humanity.